Introduction

Epigenetic regulation of neurogenesis is the role that epigenetics (hertitable characteristics that do not involve changes in DNA sequence, such as chemical modifications to DNA or histone proteins) plays in the regulation of neurogenesis (the production of neurons from neural stem cells, a process critical for brain development, learning, and memory).
Epigenetics is the study of heritable changes in gene expression (e.g., turning genes "on" or "off") which do not result from modifications to the sequence of DNA. Neurogenesis is the mechanism for neuron proliferation (the creation of new neural stem cells) and differentiation (their transformation into specialized neurons). These processes are tightly regulated and depend on precise timing and order, ensuring proper brain formation and function.[1]
For example, during brain development, neural stem cells must "decide" whether to self-renew or mature into neurons—a choice guided by epigenetic "tags" like DNA methylation or histone acetylation. A 2017 study in Nature Neuroscience demonstrated that DNA methyltransferase enzymes (DNMTs) directly silence stem cell self-renewal genes like Hes1, forcing differentiation into neurons [2].Processes such as neuron proliferation, fate specification, differentiation, maturation, and functional integration of newborn cells into existing neuronal networks are all interconnected.[3] For instance, histone deacetylases (HDACs) remove acetyl groups from histones, condensing chromatin to repress genes that maintain stemness—a process shown to be essential for neuronal maturation in a 2020 Cell Stem Cell study[4]. In the past decade[when?] many epigenetic regulatory mechanisms have been shown to play a large role in the timing and determination of neural stem cell lineages.[1] A pivotal 2018 Science paper revealed that the long non-coding RNA Pnky interacts with the RNA-binding protein PTBP1 to regulate splicing events critical for neurogenesis, linking RNA-based epigenetics to neuronal fate [5].
Mechanisms

Three important methods of epigenetic regulation include histone modification, DNA methylation and demethylation, and microRNA (miRNA) expression. Histones keep the DNA of the eukaryotic cell tightly packaged through charge interactions (like molecular "Velcro") between the positive charge on the histone tail and the negative charge of the DNA, as well as between histone tails of nearby nucleosomes. While there are many different types of histone modifications, in neural epigenetics there are two primary mechanisms which have been explored: histone methylation and histone acetylation.[1][6]
Histone Methylation:
In the former, methyl groups are either added or removed to the histone altering its structure and exposing chromatin and leading to gene activation or deactivation. For example, trimethylation of histone H3 at lysine 4 (H3K4me3) is associated with active gene promoters in neurons, while H3K27me3 (trimethylation at lysine 27) represses genes that maintain stem cell identity. A 2020 study in Cell Reports revealed that the Polycomb repressive complex 2 (PRC2), which deposits H3K27me3, silences differentiation genes like Neurog2 to preserve neural stem cell pools in the developing brain [7].
Histone Acetylation:
In the latter, histone acetylation (the addition of acetyl groups by histone acetyltransferases, HATs) neutralizes the positive charge on histones, loosening their grip on DNA and allowing transcription factors to access genes. This process is dynamically regulated: histone deacetylases (HDACs) remove acetyl groups, condensing chromatin to silence genes. A 2020 study in Neuron showed that HDAC3 maintains adult hippocampal neural stem cells by balancing quiescence and activation—deleting HDAC3 disrupts neurogenesis and impairs memory formation [8].
DNA Methylation/Demethylation:
DNA methylation, in which methyl groups are added to cytosine or adenosine residues on the DNA (forming 5-methylcytosine, typically at CpG sites), is a more lasting method of gene inactivation than histone modification, though is still reversible in some cases.[1][6] Active demethylation is mediated by Ten-Eleven Translocation (TET) enzymes, which oxidize 5-methylcytosine to promote DNA repair machinery. A 2024 Science Advances study demonstrated that TET3 oxidizes methylated DNA in adult neural stem cells, activating Prox1 to drive hippocampal neurogenesis[9]. Additionally, dynamic DNA methylation by DNMT3A in adult-born neurons is critical for their survival and integration into memory circuits, as shown in a 2020 Nature Neuroscience study[10].
MicroRNAs (miRNAs):
MicroRNAs are a small form of non-coding RNA (ncRNA) that often act as "fine-tuning" mechanisms for gene expression by repressing or degrading messenger RNA (mRNA) in neural cells. For instance, miR-9 coordinates the switch from neural progenitor proliferation to differentiation by directly repressing stem cell genes like Hes1 and Tlx, as demonstrated in a 2019 study [11] miRNAs can also act directly with transcription factors to guide neurogenesis. A 2020 PNAS paper revealed that miR-124, the most abundant brain miRNA, promotes adult neurogenesis by targeting mRNAs like PTBP1 and SCP1 to enhance neuronal maturation and synaptic integration [12].
Embryonic neurogenesis
Histone modifications
Neural stem cells are involved in the development of the cortex in a precise "inside out" manner (where older neurons form deeper cortical layers, and newer neurons migrate past them to form upper layers) with carefully controlled timing mechanisms. Early born neurons form deep layers in the cortex while newer born neurons form the upper layers. This timing program is seen in vitro as well as in vivo.[1][6]
Histone methylation directs cortical layering:
Mutant analysis revealed that disrupting the PRC2 complex, which contains the histone methyltransferase Ezh2, reduces the number of upper-layer neurons (expressing markers like POU3F2/BRN2 and SATB2) by half, without affecting deeper layers. A study confirmed that PRC2-mediated H3K27me3 silences stem cell genes like Sox11, enabling progenitors to transition into upper-layer neurons [13].
In mouse embryonic stem cell-derived neural progenitors, increased histone acetylation induced by the histone deacetylase (HDAC) inhibitor valproic acid not only induced neuronal differentiation, but also selectively enriched the upper layer neuronal population. Therefore, it has been proposed that HDAC inhibition promotes the progression of neuronal differentiation, leading to a fate-switch from deep-layer producing progenitors into upper-layer progenitors. However, the reasons behind this selective differentiation and timing control as a result of HDAC inhibition are not yet fully understood.[6]
DNA methylation
DNA methylation's critical role in corticogenesis (cerebral cortex formation) has been shown through knockout experiments in mice.
- DNMT3b and DNMT1 ablation in embryos causes lethality due to neural tube defects [14].
- DNMT3a deletion does not cause embryonic death but severely impairs postnatal neurogenesis [14].
Timing of DNMT Activity:
- DNMT3b is expressed in early neural progenitors and declines as development progresses.
- DNMT3a is barely detectable until embryonic day 10 (E10), surges from E13.5, and persists in adult neurogenic regions like the subventricular zone (SVZ) and hippocampal dentate gyrus [15].
DNMT3a Regulates Neuron-Glia Fate Switching:
Loss of DNMT3a in postnatal neural progenitors downregulates neuronal genes (Dlx2, Neurog2, Sp8) and upregulates astroglial/oligodendroglial genes. A 2021 Science Advances study confirmed that DNMT3a maintains neuronal identity by methylating promoters of gliogenic genes like Sox9 [15].
Dynamic DNA Methylation in Key Genes:
- Hes5:
- Hypermethylated at E7.5 but demethylated by E9.5 via TET enzymes (not GCM1/2).
- A 2018 Genes & Development study showed TET2 oxidizes 5-methylcytosine at Hes5, enabling Notch signaling to drive neural stem cell expansion [16].
- Gfap:
- Repressed in early neurogenesis due to methylated STAT3 binding sites.
- A 2020 Cell Reports study demonstrated DNMT3a maintains Gfap methylation until E14.5, delaying astrocyte differentiation [17].
miRNAs

Mechanism of miRNAs
Dicer Knockout Insights:
Conditional knockout of Dicer (an enzyme essential for miRNA synthesis) in the mouse neocortex reduces cortical size, increases neuronal apoptosis, and disrupts cortical layering. Neuroepithelial and progenitor cells remain unaffected until embryonic day 14 (E14), after which they undergo apoptosis. A 2019 eLife study confirmed that this stage-specific requirement for miRNAs ensures proper cortical development, though the exact miRNAs involved remain unclear [18].
Key miRNAs in Neurogenesis:
- miR-124: The most abundant CNS miRNA suppresses Sox9 to drive subventricular zone progenitors into neuroblasts. A 2020 Cell Reports study showed that miR-124 directly silences Sox9 via a conserved binding site in its 3’UTR, enabling neuronal lineage progression [19].
- miR-9: Regulates neuronal differentiation and self-renewal. A 2021 Nature Neuroscience study found that ectopic miR-9 in mouse cortex prematurely activates NeuroD1 (a pro-neuronal gene) and disrupts neuronal migration by targeting Foxg1 [20].
Beyond "Fine-Tuning":
Contrary to the idea that miRNAs only fine-tune gene expression, miR-9 and miR-124 can reprogram human fibroblasts into neurons without transcription factors like NeuroD1. A 2022 Science Advances study demonstrated that these miRNAs alone remodel chromatin accessibility at neuronal loci (e.g., MAP2, SYN1), enabling fibroblast-to-neuron conversion—albeit less efficiently than transcription factor-based methods [21].
Importantly, these epigenetic mechanisms are not exclusive to embryonic development. In adults, dynamic DNA methylation, histone modifications, and miRNAs continue to regulate neurogenesis in specialized niches like the hippocampal dentate gyrus and subventricular zone. For instance, DNMT3a-dependent methylation and miR-124 remain critical for maintaining the balance between neuronal stem cell quiescence and activation in the adult brain, ensuring lifelong plasticity and cognitive function [22][23].
Adult neurogenesis
DNA methylation

Neurogenesis that persists beyond embryonic development and through adulthood is referred to as adult neurogenesis.[3] An important animal gene involved in the epigenetic regulation of adult neurogensis is the Growth arrest and DNA-damage-inducible, beta (GADD45b) gene, often studied in rodents like mice[24]. Through the demethylation of promoters, GADD45b activates genes such as brain-derived neurotrophic factor (BDNF) and basic fibroblast growth factor (FGF2)[25] which are essential for neural progenitor cell development. Consequently when there is an upregulation of GADD45b, there is increased expression of BDNF and FGF2 resulting in more neural progenitor cells in adulthood.[1][25]
Histone Acetylation in Neural Stem Cell Regulation

DNA is packaged into chromatin, a structure made up of nucleosome subunits consisting of four histone proteins H2A, H2B, H3 and H4. Both histone acetylation and deacetylation play a role in the proliferation and self-renewal of post-embryonic neural stem cells. In the absence of acetylation, H4 histones retain their basic charge, allowing them to interact with the acidic pockets of H2A-H2B dimers in neighboring nucleosomes. This interaction reinforces nucleosome-nucleosome association, resulting in more tightly packed chromatin. Acetylation neutralizes the basic charge of H4 histones, disrupting these interactions and thereby preventing chromatin compaction.[26] This process allows for greater expression of target genes like brain-derived neurotrophic factor which are involved in adult neurogenesis. Acetylation is made possible by histone acetyltransferases (HATs), which add acetyl groups to histones, promoting gene expression by loosening chromatin structure. Conversely, histone deacetylases (HDACs) remove acetyl groups, leading to chromatin condensation and gene repression. Histone acetylation plays a key role in the differentiation of nerual stem cells into specific cell types. A notable example is the chromatin regulator BRPF1, which is abundantly expressed in the developing central nervous system. It is crucial for the formation of brain regions such as the neocortex and dentate gyrus of the hippocampus. BRPF1 functions by activating histone acetyltransferases like MOZ, MORF, and HBO1[27], which drive histone acetylation processes essential for proper neural development.
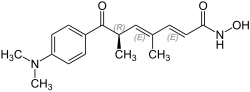
Role of HDAC Inhibitors in Promoting Neurogenesis

Histone deacetylase inhibitors (HDACi)—such as valproic acid (VPA) and trichostatin A—can enhance adult neurogenesis by blocking HDAC activity, which promotes the differentiation of adult neural progenitor cells[28]. In neural stem cells, HDAC1 and HDAC2 work with the transcription factor TLX to suppress genes that limit cell proliferation, including the cell cycle inhibitor P21 and the tumor suppressor Pten.[29] This repression supports the self-renewal and proliferation of neural stem cells. However, when HDACs are inhibited—such as by VPA, an antiepileptic drug—it can shift neural stem cells toward neuronal differentiation. Similar to processes seen in embryonic neurogenesis, VPA can also suppresses glial cell differentiation in adult neural stem cells. This effect is likely driven by the upregulation of neuron-specific genes, including neurogenic basic helix-loop-helix (bHLH) transcription factors like NEUROD, NEUROGENIN1, and MATH1. While HDAC inhibition can promote neurogenesis, complete loss of HDAC1 and HDAC2 in neural progenitor cells has the opposite effect, potentially preventing proper neuronal differentiation. Similarly, their loss in oligodendrocyte progenitor cells disrupts oligodendrocyte formation, highlighting that histone deacetylation plays distinct and essential roles at various stages of neural development.
MicroRNAs and Post-Transcriptional Regulation of Neurogenesis

MicroRNAs (miRNAs) are small noncoding RNAs that regulate gene expression at the post-transcriptional level, controlling protein production without altering the DNA sequence. One important microRNA in adult neurogenisis is miR-9, which plays a crucial role in cell differentiation.[30] miR-9 targets the nuclear receptor TLX in adult neurogenesis to promote neural differentiation and inhibit neural stem cell proliferation. It also influences neuronal subtype specification and regulates axonal growth, branching, and targeting in the central nervous system through interactions with HES1, a neural stem cell homeostasis molecule.

Another crucial miRNA in adult neurogenisis is miR-124, which promotes cell cycle exit and neuronal differentiation. Several mouse studies have shown that ectopic expression of miR-124 leads to premature differentiation and depletion of neural progenitor cells in the subventricular zone (SVZ), a major adult neurogenic region lining the lateral ventricles[31]. The SVZ continuously generates neuroblasts that migrate to the olfactory bulb, and miR-124 overexpression disrupts this process by forcing early cell cycle exit, ultimately reducing long-term neurogenesis. In addition to miR-9 and miR-124, other miRNAs play essential roles in regulation of adult neurogenesis. miR-137, miR-184 and miR-195 regulate adult neural stem cell proliferation, with their over-expression leading to up-regulated proliferation while their down-regulation leads to a decrease in neuronal proliferation[32]. Methyl-CpG binding protein 1 (MBD1) represses miR-184, a microRNA that promotes the proliferation of adult neural stem/progenitor cells (aNSCs) while inhibiting their differentiation by targeting and downregulating Numblike (Numbl), a protein involved in promoting neuronal differentiation. In adult neurogenesis, MBD1, miR-184, and Numbl function together to balance stem cell maintenance and neuronal output. Similarly, miR-195 forms a negative regulatory loop with MBD1; its inhibition promotes aNSC differentiation, and its levels naturally decrease as differentiation proceeds.[33] Disruptions in MBD1 or miRNA signaling have been linked to neurodevelopmental disorders, mood disorders, and impaired cognitive function, all of which underscore the importance of finely tuned adult neurogenesis.
Astrocyte reprogramming

Astrocytes are glial cells that form the blood brain barrier and support the synapse.[34] Unlike neurons, glial cells are can alter their cell fate prior to reaching full maturation, and"dedifferentiate” due to epigenetic factors. While dedifferentiation is still possible, the expression of genes Mash1, NeuroG1 and NeuroG2 can reprogram astrocytes into neurons.[35] This made possible by the increased acetylation at the H3K9 and H3K14 residues near the NeuroG1 and NeuroG2 genes, trigger neuron differentiation.[36]
Additionally, silencing DNA methylation mechanisms—particularly various DNA methyltransferases—prevents astrocyte progenitor cells from redifferentiating into their original glial cell fate.[37] Repression of DNA methylation is essential to block genes required for astrocyte maturation, maintaining a more plastic, undifferentiated state. Overexpression of the histone methyltransferase Ezh2, which catalyzes tri-methylation at H3K27, represses genes involved in astrocyte maintenance, allowing the cell to retain a neural stem cell-like morphology.[38] This shows that different methyltransferases can either promote or inhibit astrocyte dedifferentiation, depending on their activity. Although Ezh2 alone cannot induce dedifferentiation, it is necessary for the process, as its absence prevents astrocytes from reverting to a progenitor state. Once in this state, expression of NeuroD4 can drive neuronal differentiation, enabling neurogenesis from dedifferentiated astrocytes in the adult brain.[39]
In memory
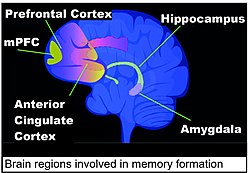
The Growth Arrest and DNA Damage inducible 45 (Gadd45) gene family plays a large role in the hippocampus. Gadd45 facilitates hippocampal long-term potentiation and enhances persisting memory for motor performance, aversive conditioning, and spatial navigation.[40] Additionally, DNA methylation has been shown to be important for activity-dependent modulation of adult neurogenesis in the hippocampus, which is mediated by GADD45b. GADD45b seems to act as a sensor in mature neurons for environmental changes which it expresses through these methylation changes.[1] This was determined by examining the effects of applying an electric stimulus to the hippocampal dentate gyrus (DG) in normal and GADD45b knockout mice. In normal mice application of electrical stimulation to the DG increased neurogenesis by increasing BDNF. However, in GADD45b deficient mice the electrical stimulus had less of an effect. Further analysis revealed that this effect is mediated through the activation of immediate early genes (IEGs), including c-Fos and Arc, which are crucial for synaptic plasticity.
Recent research has also linked GADD45b to cognitive flexibility, demonstrating that its deficiency impairs reversal learning in behavioral assays. This suggests that GADD45b influences both memory persistence and adaptability, making it a key target for neurocognitive interventions.
Further examination revealed that around 1.4% of CpG islands in DG neurons are actively methylated and demethylated upon electric shock. This shows that the post-mitotic methylation states of neurons are not static and given that electric shock equipment such as that used in the study has been shown to have therapeutic effects to human patients with depression and other psychiatric disorders, the possibility remains that epigenetic mechanisms may play an important role in the pathophysiology of neuropsychiatric disorders.[3][25]

Histone modifications have also been linked to learning and memory. Histone acetylation, particularly at H3 and H4, has been associated with increased synaptic plasticity and memory formation.[41] Studies indicate that histone deacetylase (HDAC) inhibitors, such as Trichostatin A (TSA) and sodium butyrate, enhance long-term potentiation and memory consolidation by promoting chromatin relaxation and increasing gene accessibility.[42] Additionally, histone methylation plays a role in regulating neuronal plasticity. Specifically, H3K4 methylation has been shown to activate memory-related genes, whereas H3K9 and H3K27 methylation are associated with gene repression. The balance of these modifications influences cognitive function and memory retention.[43] These modifications interact dynamically, with H3K4me3 enrichment linked to active transcription and memory consolidation, while H3K27me3-mediated repression prevents aberrant gene activation.

MicroRNAs also contribute to the epigenetic regulation of memory. miR-132, in particular, has been found to promote dendritic growth and synaptic plasticity, which are essential for learning processes.[41] Dysregulation of miR-132 has been implicated in cognitive impairments, suggesting its role in memory-related disorders.[43] Additionally, miR-124, a key regulator of neuronal differentiation, has been shown to influence synaptic function and plasticity by modulating chromatin structure and transcription factor expression.[44] Emerging evidence suggests that miR-124 also regulates neuronal excitability by targeting ion channel transcripts, further influencing learning and memory pathways.
DNMT1 and DNMT3a are both required in conjunction for learning, memory, and synaptic plasticity.[25] These enzymes contribute to the maintenance of methylation homeostasis, with DNMT3a facilitating de novo methylation of plasticity-related genes while DNMT1 ensures the stability of established epigenetic marks.
Epigenetic dysregulation and neurological disorders

Epigenetic dysregulation, or alterations in epigenomic machinery, can cause DNA methylation and histone acetylation processes to go rogue. The epigenetic machinery influences neural differentiation regulation (i.e. neurogenesis) [45] and are also involved in processes related to memory consolidation and learning in healthy individuals.[46] DNA methylation and histone modifications play a critical role in modulating gene expression related to synaptic plasticity, which is essential for learning and memory formation.[41] Increasing age can produce various epigenetic changes such as reduced global heterochromatin, nucleosome remodeling, altered histone marks, and changes in DNA methylation. For instance, nucleosome loss occurs due to aging because core histone proteins are lost and less protein synthesis occurs.[47] Epigenetic control of enhancer regions in neurons has been linked to neurodegenerative diseases, particularly Alzheimer's disease, where dysregulated chromatin accessibility contributes to neuronal dysfunction.[48] Notably, chromatin loops that regulate enhancer-promoter interactions appear to be disrupted in neurodegenerative conditions, leading to widespread transcriptional alterations. As aging is the main risk for many neurological disorders, epigenetic dysregulation can in turn lead to alterations on the transcriptional level of genes involved in the pathogenesis of neural degenerative diseases such as Parkinson's disease, Alzheimer's disease, Huntington's disease, schizophrenia, and bipolar disease.[1][49]
DNA hydroxymethylation, a modification mediated by TET enzymes, has recently been implicated in the aging brain. Studies show that global 5-hydroxymethylcytosine (5hmC) levels decline with age, potentially contributing to neurodegeneration through loss of gene activation at critical neuronal loci.

Recent studies highlight that epigenetic mutations affecting chromatin regulators can result in widespread transcriptional disruptions, contributing not only to common neurodegenerative diseases but also to rare neurological conditions.[43] For example, mutations in MECP2, a key epigenetic regulator, lead to Rett syndrome, a severe neurodevelopmental disorder characterized by intellectual disability and motor impairments.
Alzheimer's disease

MicroRNA expression is critical for neurogenesis. In patients with Alzheimer's disease miR-9 and miR-128 is upregulated, while miR-15a is downregulated.[50] These microRNAs have been shown to regulate key neuronal genes involved in synaptic plasticity and neuroinflammation, further linking their dysregulation to cognitive decline in Alzheimer's patients.[41] Alzheimer's patients also show decreases in brain-derived neurotrophic factor, which has been shown to be repressed through DNA methylation.[25] BDNF reduction impairs neuronal survival and synaptic function, exacerbating memory deficits associated with the disease.[41] Loss of BDNF signaling is associated with synaptic atrophy, contributing to the hallmark cognitive deficits of Alzheimer’s disease. Although one of the strongest pieces of evidence for epigenetic influence in Alzheimer's is the gene that controls the protein responsible for amyloid plaque formation, APP. This gene has very high GC content in its promoter region, meaning that it is highly susceptible to DNA methylation. This promoter site has been shown to naturally reduce methylation with aging, exemplifying the parallels between aging and Alzheimer's already well known.[51][52] Epigenetic regulation of enhancer regions in neurons has also been implicated in Alzheimer’s disease, with studies showing that chromatin accessibility changes contribute to disease progression by altering transcriptional programs essential for neuronal function. Additionally, recent findings suggest that differential DNA methylation of tau-related genes contributes to tau pathology, another defining feature of Alzheimer’s disease.

Heavy metals also seem to interfere with epigenetic mechanisms. Specifically in the case of APP, lead exposure earlier in life has been shown to cause a marked over-expression of the APP protein, leading to more amyloid plaque later in life in the aging brain.[52] Other environmental toxins, such as air pollutants and pesticides, have also been suggested to induce epigenetic modifications that contribute to Alzheimer's pathology.[43]
DNA methylation's age relation has been further investigated in the promoter regions of several Alzheimer's related genes in the brains of postmortem late-onset Alzheimer's disease patients. The older patients seem to have more abnormal epigenetic machinery than the younger patients, despite the fact that both had died from Alzheimer's. Though this in of itself is not conclusive evidence of anything, it has led to an age-related epigenetic drift theory where abnormalities in epigenetic machinery and exposure to certain environmental factors which occur earlier in life lead to aberrant DNA methylation patterns far later, contributing to sporadic Alzheimer's Disease predisposition.[52] This epigenetic drift theory suggests that cumulative changes in DNA methylation, histone modifications, and chromatin remodeling over time contribute to neurodegeneration, providing a potential link between aging and late-onset Alzheimer’s disease.[43]

Histone modifications may also have an impact in Alzheimer's disease, but the differences between HDAC effects in rodent brains compared to human brains have researchers puzzled.[52] As the focus for neurodegenerative diseases begins to shift towards epigenetic pharmacology, it can be expected that the interactions of histone modifications with respect to neurogenesis will become more clear. Histone deacetylase (HDAC) inhibitors have shown promise in preclinical studies, as they can restore cognitive function by enhancing synaptic plasticity and memory consolidation, though their effects in humans remain under investigation.[43]

Huntington’s disease
Histone acetylation has received increasing support over the years as a proposed mechanism through which epigenetic dysregulation leads changes in gene expression that contribute to HD.[53] Studies that look at mice with HD versus the wild type (WT) have shown that specific gene loci (Drd2, Penk1, Actb, and Grin1) decrease in histone acetylation levels, suggesting that a mutation of the Huntington (HTT) gene and its overexpression may be the cause of this epigenetic dysregulation. Additionally, research has demonstrated that mutant HTT can interfere with histone acetyltransferase (HAT) activity, further reducing histone acetylation and leading to widespread transcriptional repression in neurons.[41]
It has been thought that HDAC inhibitors (HDACi's) could partially reverse the low acetylation levels seen in patients with HD. Preclinical studies have been performed using various HDACi's [such as suberoxylanilide hydroxamic acid (SAHA), Trichostatin A (TSA), phenylbutyrate, and sodium butyrate (NaB)] that target HDACI and HDACII. Although these inhibitors improve some phenotypes of HD in mice, such as neuropathology and motor function, these beneficial effects do not lead towards a conclusion for the definitive need for increasing acetylation levels in HD patients. Recent findings suggest that HDAC inhibition may not only affect histone proteins but also modulate non-histone targets involved in neuronal survival, axonal transport, and protein aggregation.[43]
However, inactivation of a target of SAHA, Hdac 4, alleviates neurodegenerative complications in mice with HD through a transcription-independent mechanism which acts upon mutant Htt aggregation processes-which may indicate that there is a mechanism involving non-histone proteins.[54] The proposed mechanism through which SAHA is speculated to act is through a RANBP2-mediated proteasome degradation model, where HDAC inhibition promotes enhanced clearance of misfolded mutant HTT aggregates.[48] In this mechanism, SAHA is shown to down-regulate Hdac 4 through an increase in sumoylation, which is then followed up with the activation of degradation through a proteasomal pathway. This mechanism reveals the connectivity between acetylation, deacetylation, and sumoylation processes.[55]

As of 2014, HDACi treatment has not been shown to restore normal expression of neuronal-identity genes.[56] However clinical studies using HDACi are currently ongoing and the results are pending, with the Phase II studies showing promise for safe and tolerable use of several compounds such as phenylbutyrate. Newer approaches are investigating more selective HDAC inhibitors that target specific isoforms, aiming to minimize off-target effects while maximizing therapeutic benefits.[43]
Non-histone-mediated beneficial effects of HDACi have also been documented in models of Parkinson disease, suggesting common mechanisms between several neurodegenerative diseases. This overlap highlights the potential of epigenetic-based therapies to target multiple neurodegenerative conditions by restoring transcriptional homeostasis and reducing toxic protein accumulation.[43]

Parkinson’s disease
DNA methylation analysis showed that there is significant dysregulation of methylation on CpG islands in patients with PD when compared to healthy individuals. Although this was genome-wide, this also occurred on many PD risk genes.[57] Specifically, differential methylation patterns have been identified in genes associated with dopaminergic neuron survival, inflammation, and mitochondrial function, highlighting epigenetic regulation as a key factor in PD pathogenesis.[41]
Mitochondrial DNA methylation of cytosine has also been shown to fluctuate over time due to age variance, as there is a growing body of literature linking mtDNA methylation to aging and oxidative stress.[58] A study from 2015 by Hashizume et al. showed that SHMT2 mRNA levels are significantly reduced in the fibroblasts of old people when compared to younger individuals. The study also further indicated that decreased GCAT and SHMT2 levels of gene expression via shRNA and siRNA, respectively, in the fibroblasts of young patients led to a respiratory chain dysfunction typical for senile individuals-suggesting that an epigenetic mechanism may be the cause for the phenotypic change. These findings reinforce the role of mitochondrial epigenetics in cellular aging and suggest that PD-related mitochondrial dysfunction may, in part, be driven by epigenetic modifications.[43] As mitochondria plays a role in the development of the PD,[59] further research into the area will help uncover any implications that mitochondrial DNA methylation plays in the pathogenesis of PD.

The use of dopaminergic neurons that have been isolated from the PD patients indicated that there were increases in acetylation (at H2A, H3 and H4) when compared to the age-control group.[57] Histone acetylation changes have been linked to transcriptional dysregulation in PD, affecting genes responsible for neuronal survival and synaptic plasticity.[41] Another study involving MPP+ (a compound that can cause a disease state resembling mammals and humans with PD[60])-treated cells and (MPP+)-treated mouse brains showed decreased HDAC levels, as well as in midbrain samples from patients with PD. This is seen potentially due to how MPP+ promotes the breakdown of HDAC1 and HDAC2 via autophagy, a bodily process of cycling out old cells to make room for newer, healthier cells.[61] These results point toward the stress of histone modifications in regard to chromatin remodeling and its implication in the pathogenesis of PD. Further, altered histone deacetylation has been shown to affect key pathways involved in neuroinflammation and dopaminergic neuron survival, contributing to disease progression.[43]
miRNAs are also emerging as relevant contributors to neurodegeneration in PD. In particular, the frontal cortex PD patients have shown higher levels of LRRK2 and lower levels of miR-205 when compared to healthy individuals. Connecting this to the findings of miR-205's ability to bind to the 3′ UTR of LRRK2 mRNA and suppress expression, as well as miR-205's prevention of defects after introduction to a R1441G LRRK2 mutation, these results point towards miR-205 and its regulatory role in LRRK2 expression-which in turn suggest a regulatory role in the pathogenesis of PD. This highlights the therapeutic potential of miRNA-based interventions aimed at restoring normal gene expression in PD patients.[48]
In another study in which increasing microtubule acetylation using deacetylase inhibitors or the tubulin acetylase αTAT1 showed prevention of the association of mutant LRRK2 with microtubules, inhibition of deacetylases HDAC6 and Sirt2 through knockdown processes rescued both axonal transport and locomotor behavior.[62] This further connects to the common mechanisms involving HDACi in various neurodegenerative diseases. Targeting HDAC6 and Sirt2 has been proposed as a potential neuroprotective strategy, as these enzymes regulate cellular stress responses and cytoskeletal stability in neurons.[43]
Bipolar Disorder
Bipolar disorders are both highly complex and heritable, which makes it an interesting disorder to examine for epigenetic modifications. DNA methylation, DNA hydroxymethylation, and histone modifications are all capable of contributing to the formation of bipolar disorder. Epigenetic mechanisms can influence key neurotransmitter systems, neuroinflammatory pathways, and circadian rhythm genes, all of which are implicated in bipolar disorder pathophysiology.[41]

For example, studies of monozygotic twins revealed that individuals with bipolar disorder had lower methylation of the peptidylprolyl isomerase E-like (PPIEL) gene, which can be attributed to the dopamine transmission. The studies indicated that hypermethylation of SLC6A4, a serotonin transporter gene, is also involved with bipolar disorder. Altered serotonin transporter methylation has been linked to mood instability and antidepressant response in affected individuals.[41] Greater expression of DNA methyltransferase 1 in cortical GABAergic interneurons may enable hypermethylation. Hypermethylation may prompt hydroxymethylation to occur in order to overcompensate for the repressive effects of hypermethylation.
The methylation of CpG regions are relevant to bipolar disorders. Patients with bipolar disorder showed lower methylation levels for the CpG region of the KCNQ3 gene, which is responsible for the voltage-gated K+ channel. Since voltage-gated potassium channels regulate neuronal excitability, their dysregulation could contribute to the manic and depressive episodes characteristic of bipolar disorder.[43] Childhood maltreatment contributed to the methylation status of CpG2 III of 5-hydroxytryptamine 3A, which alters how maltreatment affects bipolar disorder. These findings suggest that early-life stressors can leave lasting epigenetic marks that modulate the risk and severity of bipolar disorder later in life.[43]

Moreover, therapeutic interventions such as engineered transcription factors could modify chromatin structure to address the epigenetic changes found in those with bipolar disorder. DNA methyltransferase (DNMT) inhibitors and histone deacetylase (HDAC) inhibitors could possibly reverse epigenetic modifications in order to therapeutically address bipolar disorder. HDAC inhibitors have been shown to regulate gene expression patterns involved in mood stabilization, and preclinical studies suggest they may enhance the efficacy of conventional mood stabilizers such as lithium.[41] DNMT inhibitors and HDAC often produces antidepressant-like effects. However, challenges remain in developing targeted epigenetic therapies that can selectively modify aberrant epigenetic marks without widespread off-target effects.[43]
Epigenetic therapies for neurodegenerative and psychiatric disorders

Given the growing evidence of epigenetic involvement in neurological and psychiatric disorders, researchers are investigating epigenetic-based therapies. HDAC inhibitors, such as valproic acid and vorinostat, have been explored as potential treatments for Alzheimer’s, Parkinson’s, and Huntington’s diseases due to their ability to enhance gene expression related to neuronal survival and synaptic plasticity.[43]
Similarly, DNA methylation inhibitors, such as 5-azacytidine and RG108, are being investigated for their potential to reverse hypermethylation of critical genes in disorders like schizophrenia and Alzheimer’s disease. However, challenges remain in developing targeted epigenetic therapies that selectively modify disease-relevant genes without causing widespread epigenetic disruption.
MicroRNA-based therapies are also under exploration, with strategies including miRNA mimics to restore deficient microRNAs or miRNA inhibitors (antagomirs) to suppress overexpressed microRNAs in disease contexts. For example, miR-132 mimics have shown promise in restoring synaptic function in Alzheimer’s disease models, while miR-137 modulators are being explored for potential schizophrenia treatments.
Conclusion
Epigenetic mechanisms, including DNA methylation, histone modifications, and microRNA regulation, play crucial roles in neural development, memory formation, and neurodegeneration. Dysregulation of these processes is increasingly recognized as a key contributor to neurological and psychiatric disorders, including Alzheimer’s disease, schizophrenia, Parkinson’s disease, and Huntington’s disease. Advancing our understanding of these epigenetic changes may lead to novel diagnostic biomarkers and therapeutic interventions, paving the way for more effective treatments for these debilitating conditions.
References
- ^ a b c d e f g h Hu XL, Wang Y, Shen Q (April 2012). "Epigenetic control on cell fate choice in neural stem cells". Protein & Cell. 3 (4): 278–290. doi:10.1007/s13238-012-2916-6. PMC 4729703. PMID 22549586.
- ^ Siclari, Francesca; Baird, Benjamin; Perogamvros, Lampros; Bernardi, Giulio; LaRocque, Joshua J.; Riedner, Brady; Boly, Melanie; Postle, Bradley R.; Tononi, Giulio (June 2017). "The neural correlates of dreaming". Nature Neuroscience. 20 (6): 872–878. doi:10.1038/nn.4545. ISSN 1546-1726. PMC 5462120. PMID 28394322.
- ^ a b c Faigle R, Song H (February 2013). "Signaling mechanisms regulating adult neural stem cells and neurogenesis". Biochimica et Biophysica Acta (BBA) - General Subjects. 1830 (2): 2435–2448. doi:10.1016/j.bbagen.2012.09.002. PMC 3541438. PMID 22982587.
- ^ Shook, Brett A.; Wasko, Renee R.; Mano, Omer; Rutenberg-Schoenberg, Michael; Rudolph, Michael C.; Zirak, Bahar; Rivera-Gonzalez, Guillermo C.; López-Giráldez, Francesc; Zarini, Simona; Rezza, Amélie; Clark, Damon A.; Rendl, Michael; Rosenblum, Michael D.; Gerstein, Mark B.; Horsley, Valerie (2020-06-04). "Dermal Adipocyte Lipolysis and Myofibroblast Conversion Are Required for Efficient Skin Repair". Cell Stem Cell. 26 (6): 880–895.e6. doi:10.1016/j.stem.2020.03.013. ISSN 1934-5909. PMC 7853423. PMID 32302523.
- ^ Benjamin, Kynon J. M.; Chen, Qiang; Jaffe, Andrew E.; Stolz, Joshua M.; Collado-Torres, Leonardo; Huuki-Myers, Louise A.; Burke, Emily E.; Arora, Ria; Feltrin, Arthur S.; Barbosa, André Rocha; Radulescu, Eugenia; Pergola, Giulio; Shin, Joo Heon; Ulrich, William S.; Deep-Soboslay, Amy (November 2022). "Analysis of the caudate nucleus transcriptome in individuals with schizophrenia highlights effects of antipsychotics and new risk genes". Nature Neuroscience. 25 (11): 1559–1568. doi:10.1038/s41593-022-01182-7. hdl:11586/476780. ISSN 1546-1726. PMC 10599288. PMID 36319771.
- ^ a b c d MuhChyi C, Juliandi B, Matsuda T, Nakashima K (October 2013). "Epigenetic regulation of neural stem cell fate during corticogenesis". International Journal of Developmental Neuroscience. 31 (6): 424–433. doi:10.1016/j.ijdevneu.2013.02.006. PMID 23466416. S2CID 205242753.
- ^ Narayan, Priyanka; Sienski, Grzegorz; Bonner, Julia M.; Lin, Yuan-Ta; Seo, Jinsoo; Baru, Valeriya; Haque, Aftabul; Milo, Blerta; Akay, Leyla A.; Graziosi, Agnese; Freyzon, Yelena; Landgraf, Dirk; Hesse, William R.; Valastyan, Julie; Barrasa, M. Inmaculada (2020-10-06). "PICALM Rescues Endocytic Defects Caused by the Alzheimer's Disease Risk Factor APOE4". Cell Reports. 33 (1). doi:10.1016/j.celrep.2020.108224. ISSN 2211-1247. PMC 8190562. PMID 33027662.
- ^ Huang, Anna Yu-Szu; Woo, Junsung; Sardar, Debosmita; Lozzi, Brittney; Huerta, Navish A. Bosquez; Lin, Chia-Ching John; Felice, Daniela; Jain, Antrix; Paulucci-Holthauzen, Adriana; Deneen, Benjamin (2020-06-17). "Region-Specific Transcriptional Control of Astrocyte Function Oversees Local Circuit Activities". Neuron. 106 (6): 992–1008.e9. doi:10.1016/j.neuron.2020.03.025. ISSN 0896-6273. PMC 7879989. PMID 32320644.
- ^ MacArthur, Ian C.; Ma, Liyang; Huang, Cheng-Yen; Bhavsar, Hrutvik; Suzuki, Masako; Dawlaty, Meelad M. (2024-08-28). "Developmental DNA demethylation is a determinant of neural stem cell identity and gliogenic competence". Science Advances. 10 (35): eado5424. doi:10.1126/sciadv.ado5424. PMC 11352921. PMID 39196941.
- ^ Nagy, Corina; Maitra, Malosree; Tanti, Arnaud; Suderman, Matthew; Théroux, Jean-Francois; Davoli, Maria Antonietta; Perlman, Kelly; Yerko, Volodymyr; Wang, Yu Chang; Tripathy, Shreejoy J.; Pavlidis, Paul; Mechawar, Naguib; Ragoussis, Jiannis; Turecki, Gustavo (June 2020). "Single-nucleus transcriptomics of the prefrontal cortex in major depressive disorder implicates oligodendrocyte precursor cells and excitatory neurons". Nature Neuroscience. 23 (6): 771–781. doi:10.1038/s41593-020-0621-y. ISSN 1546-1726.
- ^ Marangon, Davide; Raffaele, Stefano; Fumagalli, Marta; Lecca, Davide (2019-10-01). "MicroRNAs change the games in central nervous system pharmacology". Biochemical Pharmacology. 168: 162–172. doi:10.1016/j.bcp.2019.06.019. hdl:2434/706752. ISSN 0006-2952. PMID 31251938.
- ^ Cheng, Li-Chun; Pastrana, Erika; Tavazoie, Masoud; Doetsch, Fiona (April 2009). "miR-124 regulates adult neurogenesis in the subventricular zone stem cell niche". Nature Neuroscience. 12 (4): 399–408. doi:10.1038/nn.2294. ISSN 1546-1726. PMC 2766245. PMID 19287386.
- ^ Toskas, Konstantinos; Yaghmaeian-Salmani, Behzad; Skiteva, Olga; Paslawski, Wojciech; Gillberg, Linda; Skara, Vasiliki; Antoniou, Irene; Södersten, Erik; Svenningsson, Per; Chergui, Karima; Ringnér, Markus; Perlmann, Thomas; Holmberg, Johan (2022-08-26). "PRC2-mediated repression is essential to maintain identity and function of differentiated dopaminergic and serotonergic neurons". Science Advances. 8 (34): eabo1543. Bibcode:2022SciA....8O1543T. doi:10.1126/sciadv.abo1543. PMC 9417181. PMID 36026451.
- ^ a b Uysal, Fatma; Ozturk, Saffet; Akkoyunlu, Gokhan (2017-12-01). "DNMT1, DNMT3A and DNMT3B proteins are differently expressed in mouse oocytes and early embryos". Journal of Molecular Histology. 48 (5): 417–426. doi:10.1007/s10735-017-9739-y. ISSN 1567-2387. PMID 29027601.
- ^ a b He, Yupeng; Hariharan, Manoj; Gorkin, David U.; Dickel, Diane E.; Luo, Chongyuan; Castanon, Rosa G.; Nery, Joseph R.; Lee, Ah Young; Zhao, Yuan; Huang, Hui; Williams, Brian A.; Trout, Diane; Amrhein, Henry; Fang, Rongxin; Chen, Huaming (July 2020). "Spatiotemporal DNA methylome dynamics of the developing mouse fetus". Nature. 583 (7818): 752–759. Bibcode:2020Natur.583..752H. doi:10.1038/s41586-020-2119-x. ISSN 1476-4687. PMID 32728242.
- ^ Yu, Tingting; Liu, Dawei; Zhang, Ting; Zhou, Yanheng; Shi, Songtao; Yang, Ruili (2019-10-14). "Inhibition of Tet1- and Tet2-mediated DNA demethylation promotes immunomodulation of periodontal ligament stem cells". Cell Death & Disease. 10 (10): 1–11. doi:10.1038/s41419-019-2025-z. ISSN 2041-4889.
- ^ Takizawa, Takumi; Nakashima, Kinichi; Namihira, Masakazu; Ochiai, Wataru; Uemura, Atsumi; Yanagisawa, Makoto; Fujita, Naoyuki; Nakao, Mitsuyoshi; Taga, Tetsuya (2001-12-01). "DNA Methylation Is a Critical Cell-Intrinsic Determinant of Astrocyte Differentiation in the Fetal Brain". Developmental Cell. 1 (6): 749–758. doi:10.1016/S1534-5807(01)00101-0. ISSN 1534-5807. PMID 11740937.
- ^ Saurat, Nathalie; Andersson, Therese; Vasistha, Navneet A.; Molnar, Zoltan; Livesey, Frederick J. (2013-07-29). "Dicer is required for neural stem cell multipotency and lineage progression during cerebral cortex development". Neural Development. 8 (1): 14. doi:10.1186/1749-8104-8-14. ISSN 1749-8104. PMC 3737057. PMID 23895693.
- ^ Cheng, Li-Chun; Pastrana, Erika; Tavazoie, Masoud; Doetsch, Fiona (April 2009). "miR-124 regulates adult neurogenesis in the subventricular zone stem cell niche". Nature Neuroscience. 12 (4): 399–408. doi:10.1038/nn.2294. ISSN 1546-1726. PMC 2766245. PMID 19287386.
- ^ Radhakrishnan, Balachandar; Anand, A. Alwin Prem (2016-01-01). "Role of miRNA-9 in Brain Development". Journal of Experimental Neuroscience. 10: 101–120. doi:10.4137/JEN.S32843. ISSN 1179-0695. PMC 5053108. PMID 27721656.
- ^ Faravelli, Irene; Corti, Stefania (2018-05-01). "MicroRNA-Directed Neuronal Reprogramming as a Therapeutic Strategy for Neurological Diseases". Molecular Neurobiology. 55 (5): 4428–4436. doi:10.1007/s12035-017-0671-7. ISSN 1559-1182. PMID 28664454.
- ^ Kupke, Janina; Klimmt, Julien; Mudlaff, Franziska; Schwab, Maximilian; Lutsik, Pavlo; Plass, Christoph; Sticht, Carsten; Oliveira, Ana M. M. (September 2024). "Dnmt3a1 regulates hippocampus-dependent memory via the downstream target Nrp1". Neuropsychopharmacology. 49 (10): 1528–1539. doi:10.1038/s41386-024-01843-0. ISSN 1740-634X. PMC 11319347. PMID 38499720.
- ^ Jiao, Shujie; Liu, Yaling; Yao, Yaobing; Teng, Junfang (2017-12-11). "miR-124 promotes proliferation and differentiation of neuronal stem cells through inactivating Notch pathway". Cell & Bioscience. 7 (1): 68. doi:10.1186/s13578-017-0194-y. ISSN 2045-3701. PMC 5725914. PMID 29238518.
- ^ Park, Se-Ra; Jung, Da-Young; Kim, Tae-Won; Lee, Chul-Ho; Jung, Ju-Young (2018-10-15). "Growth arrest and DNA-damage-inducible 45 beta (GADD45β) deletion suppresses testosterone-induced prostate hyperplasia in mice". Life Sciences. 211: 74–80. doi:10.1016/j.lfs.2018.09.014. ISSN 0024-3205. PMID 30195037.
- ^ a b c d e Lv J, Xin Y, Zhou W, Qiu Z (July 2013). "The epigenetic switches for neural development and psychiatric disorders". Journal of Genetics and Genomics = Yi Chuan Xue Bao. 40 (7): 339–346. doi:10.1016/j.jgg.2013.04.007. PMID 23876774.
- ^ Pepenella S, Murphy KJ, Hayes JJ (September 2014). "A distinct switch in interactions of the histone H4 tail domain upon salt-dependent folding of nucleosome arrays". The Journal of Biological Chemistry. 289 (39): 27342–27351. doi:10.1074/jbc.M114.595140. PMC 4175364. PMID 25122771.
- ^ Mishima, Yuta; Miyagi, Satoru; Saraya, Atsunori; Negishi, Masamitsu; Endoh, Mitsuhiro; Endo, Takaho A.; Toyoda, Tetsuro; Shinga, Jun; Katsumoto, Takuo; Chiba, Tetsuhiro; Yamaguchi, Naoto; Kitabayashi, Issay; Koseki, Haruhiko; Iwama, Atsushi (2011-09-01). "The Hbo1-Brd1/Brpf2 complex is responsible for global acetylation of H3K14 and required for fetal liver erythropoiesis". Blood. 118 (9): 2443–2453. doi:10.1182/blood-2011-01-331892. ISSN 0006-4971. PMID 21753189.
- ^ Hsieh, Jenny; Nakashima, Kinichi; Kuwabara, Tomoko; Mejia, Eunice; Gage, Fred H. (2004-11-23). "Histone deacetylase inhibition-mediated neuronal differentiation of multipotent adult neural progenitor cells". Proceedings of the National Academy of Sciences of the United States of America. 101 (47): 16659–16664. Bibcode:2004PNAS..10116659H. doi:10.1073/pnas.0407643101. ISSN 0027-8424. PMC 527137. PMID 15537713.
- ^ Lilja, Tobias; Heldring, Nina; Hermanson, Ola (2013-02-01). "Like a rolling histone: Epigenetic regulation of neural stem cells and brain development by factors controlling histone acetylation and methylation". Biochimica et Biophysica Acta (BBA) - General Subjects. Biochemistry of Stem Cells. 1830 (2): 2354–2360. doi:10.1016/j.bbagen.2012.08.011. ISSN 0304-4165. PMID 22986149.
- ^ Coolen, Marion; Katz, Shauna; Bally-Cuif, Laure (2013-11-20). "miR-9: a versatile regulator of neurogenesis". Frontiers in Cellular Neuroscience. 7: 220. doi:10.3389/fncel.2013.00220. ISSN 1662-5102. PMC 3834235. PMID 24312010.
- ^ Åkerblom, Malin; Sachdeva, Rohit; Barde, Isabelle; Verp, Sonia; Gentner, Bernhard; Trono, Didier; Jakobsson, Johan (2012-06-27). "MicroRNA-124 is a subventricular zone neuronal fate determinant". The Journal of Neuroscience: The Official Journal of the Society for Neuroscience. 32 (26): 8879–8889. doi:10.1523/JNEUROSCI.0558-12.2012. ISSN 1529-2401. PMC 4434222. PMID 22745489.
- ^ Szulwach KE, Li X, Smrt RD, Li Y, Luo Y, Lin L, et al. (April 2010). "Cross talk between microRNA and epigenetic regulation in adult neurogenesis". The Journal of Cell Biology. 189 (1): 127–141. doi:10.1083/jcb.200908151. PMC 2854370. PMID 20368621.
- ^ Liu C, Teng ZQ, McQuate AL, Jobe EM, Christ CC, von Hoyningen-Huene SJ, et al. (2013-01-17). Van Wijnen A (ed.). "An epigenetic feedback regulatory loop involving microRNA-195 and MBD1 governs neural stem cell differentiation". PLOS ONE. 8 (1): e51436. Bibcode:2013PLoSO...851436L. doi:10.1371/journal.pone.0051436. PMC 3547917. PMID 23349673.
- ^ Blackburn D, Sargsyan S, Monk PN, Shaw PJ (September 2009). "Astrocyte function and role in motor neuron disease: a future therapeutic target?". Glia. 57 (12): 1251–1264. doi:10.1002/glia.20848. PMID 19373940. S2CID 205833357.
- ^ Berninger B, Costa MR, Koch U, Schroeder T, Sutor B, Grothe B, Götz M (August 2007). "Functional properties of neurons derived from in vitro reprogrammed postnatal astroglia". The Journal of Neuroscience. 27 (32): 8654–8664. doi:10.1523/JNEUROSCI.1615-07.2007. PMC 6672931. PMID 17687043.
- ^ Sofroniew, Michael V.; Vinters, Harry V. (2010-01-01). "Astrocytes: biology and pathology". Acta Neuropathologica. 119 (1): 7–35. doi:10.1007/s00401-009-0619-8. ISSN 1432-0533. PMC 2799634. PMID 20012068.
- ^ Bulstrode H, Johnstone E, Marques-Torrejon MA, Ferguson KM, Bressan RB, Blin C, et al. (April 2017). "Elevated FOXG1 and SOX2 in glioblastoma enforces neural stem cell identity through transcriptional control of cell cycle and epigenetic regulators". Genes & Development. 31 (8): 757–773. doi:10.1101/gad.293027.116. PMC 5435889. PMID 28465359.
- ^ Zhang, Sheng; Kim, Bokyung; Zhu, Xiaoqing; Gui, Xuehong; Wang, Yan; Lan, Zhaohui; Prabhu, Preeti; Fond, Kenneth; Wang, Aijun; Guo, Fuzheng (2020-04-24). "Glial type specific regulation of CNS angiogenesis by HIFα-activated different signaling pathways". Nature Communications. 11 (1): 2027. Bibcode:2020NatCo..11.2027Z. doi:10.1038/s41467-020-15656-4. ISSN 2041-1723. PMC 7181614. PMID 32332719.
- ^ Griffiths BB, Bhutani A, Stary CM (June 2020). "Adult neurogenesis from reprogrammed astrocytes". Neural Regeneration Research. 15 (6): 973–979. doi:10.4103/1673-5374.270292. PMC 7034263. PMID 31823866.
- ^ Sultan FA, Wang J, Tront J, Liebermann DA, Sweatt JD (November 2012). "Genetic deletion of Gadd45b, a regulator of active DNA demethylation, enhances long-term memory and synaptic plasticity". The Journal of Neuroscience. 32 (48): 17059–17066. doi:10.1523/JNEUROSCI.1747-12.2012. PMC 3518911. PMID 23197699.
- ^ a b c d e f g h i j k Kim, Somi; Kaang, Bong-Kiun (January 2017). "Epigenetic regulation and chromatin remodeling in learning and memory". Experimental & Molecular Medicine. 49 (1): e281. doi:10.1038/emm.2016.140. ISSN 2092-6413.
- ^ "Histone Deacetylase Inhibitors Enhance Memory and Synaptic Plasticity via CREB: CBP-Dependent Transcriptional Activation - PMC". web.archive.org. 2024-12-23. Retrieved 2025-03-22.
- ^ a b c d e f g h i j k l m n o p Grezenko, Han; Ekhator, Chukwuyem; Nwabugwu, Nkechi U.; Ganga, Harshita; Affaf, Maryam; Abdelaziz, Ali M.; Rehman, Abdur; Shehryar, Abdullah; Abbasi, Fatima A.; Bellegarde, Sophia B.; Khaliq, Abdul Saboor; Grezenko, Han; Ekhator, Chukwuyem; Nwabugwu, Nkechi U.; Ganga, Harshita (2023-08-23). "Epigenetics in Neurological and Psychiatric Disorders: A Comprehensive Review of Current Understanding and Future Perspectives". Cureus. 15 (8): e43960. doi:10.7759/cureus.43960. ISSN 2168-8184. PMC 10446850. PMID 37622055.
- ^ Makeyev, Eugene V.; Zhang, Jiangwen; Carrasco, Monica A.; Maniatis, Tom (2007-08-03). "The MicroRNA miR-124 promotes neuronal differentiation by triggering brain-specific alternative pre-mRNA splicing". Molecular Cell. 27 (3): 435–448. doi:10.1016/j.molcel.2007.07.015. ISSN 1097-2765. PMC 3139456. PMID 17679093.
- ^ Yao B, Christian KM, He C, Jin P, Ming GL, Song H (September 2016). "Epigenetic mechanisms in neurogenesis". Nature Reviews. Neuroscience. 17 (9): 537–549. doi:10.1038/nrn.2016.70. PMC 5610421. PMID 27334043.
- ^ Delgado-Morales R, Agís-Balboa RC, Esteller M, Berdasco M (2017-06-29). "Epigenetic mechanisms during ageing and neurogenesis as novel therapeutic avenues in human brain disorders". Clinical Epigenetics. 9 (1): 67. doi:10.1186/s13148-017-0365-z. PMC 5493012. PMID 28670349.
- ^ Kane AE, Sinclair DA (February 2019). "Epigenetic changes during aging and their reprogramming potential". Critical Reviews in Biochemistry and Molecular Biology. 54 (1): 61–83. doi:10.1080/10409238.2019.1570075. PMC 6424622. PMID 30822165.
- ^ a b c Li, Peipei; Marshall, Lee; Oh, Gabriel; Jakubowski, Jennifer L.; Groot, Daniel; He, Yu; Wang, Ting; Petronis, Arturas; Labrie, Viviane (2019-05-21). "Epigenetic dysregulation of enhancers in neurons is associated with Alzheimer's disease pathology and cognitive symptoms". Nature Communications. 10 (1): 2246. Bibcode:2019NatCo..10.2246L. doi:10.1038/s41467-019-10101-7. ISSN 2041-1723. PMC 6529540. PMID 31113950.
- ^ Dempster EL, Pidsley R, Schalkwyk LC, Owens S, Georgiades A, Kane F, et al. (December 2011). "Disease-associated epigenetic changes in monozygotic twins discordant for schizophrenia and bipolar disorder". Human Molecular Genetics. 20 (24): 4786–4796. doi:10.1093/hmg/ddr416. PMC 3221539. PMID 21908516.
- ^ Ji F, Lv X, Jiao J (February 2013). "The role of microRNAs in neural stem cells and neurogenesis". Journal of Genetics and Genomics = Yi Chuan Xue Bao. 40 (2): 61–66. doi:10.1016/j.jgg.2012.12.008. PMID 23439404.
- ^ Balazs R, Vernon J, Hardy J (July 2011). "Epigenetic mechanisms in Alzheimer's disease: progress but much to do". Neurobiology of Aging. 32 (7): 1181–1187. doi:10.1016/j.neurobiolaging.2011.02.024. PMID 21669333. S2CID 20303624.
- ^ a b c d Daniilidou M, Koutroumani M, Tsolaki M (2011). "Epigenetic mechanisms in Alzheimer's disease". Current Medicinal Chemistry. 18 (12): 1751–1756. doi:10.2174/092986711795496872. PMID 21466476.
- ^ Francelle L, Lotz C, Outeiro T, Brouillet E, Merienne K (2017-01-30). "Contribution of Neuroepigenetics to Huntington's Disease". Frontiers in Human Neuroscience. 11: 17. doi:10.3389/fnhum.2017.00017. PMC 5276857. PMID 28194101.
- ^ Mielcarek M, Benn CL, Franklin SA, Smith DL, Woodman B, Marks PA, Bates GP (2011-11-28). "SAHA decreases HDAC 2 and 4 levels in vivo and improves molecular phenotypes in the R6/2 mouse model of Huntington's disease". PLOS ONE. 6 (11): e27746. Bibcode:2011PLoSO...627746M. doi:10.1371/journal.pone.0027746. PMC 3225376. PMID 22140466.
- ^ Scognamiglio A, Nebbioso A, Manzo F, Valente S, Mai A, Altucci L (October 2008). "HDAC-class II specific inhibition involves HDAC proteasome-dependent degradation mediated by RANBP2". Biochimica et Biophysica Acta (BBA) - Molecular Cell Research. 1783 (10): 2030–2038. doi:10.1016/j.bbamcr.2008.07.007. PMID 18691615.
- ^ Coppedè F (2014). "The potential of epigenetic therapies in neurodegenerative diseases". Frontiers in Genetics. 5: 220. doi:10.3389/fgene.2014.00220. PMC 4094885. PMID 25071843.
- ^ a b Pavlou MA, Outeiro TF (2017). "Epigenetics in Parkinson's Disease". In Delgado-Morales R (ed.). Neuroepigenomics in Aging and Disease. Advances in Experimental Medicine and Biology. Vol. 978. Cham: Springer International Publishing. pp. 363–390. doi:10.1007/978-3-319-53889-1_19. ISBN 978-3-319-53889-1. PMID 28523556.
- ^ Zinovkina LA, Zinovkin RA (December 2015). "DNA Methylation, Mitochondria, and Programmed Aging". Biochemistry. Biokhimiia. 80 (12): 1571–1577. doi:10.1134/S0006297915120044. PMID 26638681. S2CID 17044164.
- ^ Mhyre TR, Boyd JT, Hamill RW, Maguire-Zeiss KA (2012). "Parkinson's Disease". Protein Aggregation and Fibrillogenesis in Cerebral and Systemic Amyloid Disease. Subcellular Biochemistry. Vol. 65. pp. 389–455. doi:10.1007/978-94-007-5416-4_16. ISBN 978-94-007-5415-7. PMC 4372387. PMID 23225012.
- ^ Ito K, Eguchi Y, Imagawa Y, Akai S, Mochizuki H, Tsujimoto Y (2017-02-27). "MPP+ induces necrostatin-1- and ferrostatin-1-sensitive necrotic death of neuronal SH-SY5Y cells". Cell Death Discovery. 3: 17013. doi:10.1038/cddiscovery.2017.13. PMC 5327502. PMID 28250973.
- ^ Park G, Tan J, Garcia G, Kang Y, Salvesen G, Zhang Z (February 2016). "Regulation of Histone Acetylation by Autophagy in Parkinson Disease". The Journal of Biological Chemistry. 291 (7): 3531–3540. doi:10.1074/jbc.M115.675488. PMC 4751393. PMID 26699403.
- ^ Godena VK, Brookes-Hocking N, Moller A, Shaw G, Oswald M, Sancho RM, et al. (October 2014). "Increasing microtubule acetylation rescues axonal transport and locomotor deficits caused by LRRK2 Roc-COR domain mutations". Nature Communications. 5: 5245. Bibcode:2014NatCo...5.5245G. doi:10.1038/ncomms6245. PMC 4208097. PMID 25316291.
External links
- Database of known microRNAs: http://www.miRbase.org
- Article on histone methylation visualization through fluorescent imaging